To develop batteries with high energy density, Li metal anodes and Ni-rich cathodes are considered desirable candidates. To solve these problems with Li-metal batteries (LMBs), lithium difluoro(bisoxalato)phosphate (LiDFBP) and lithium nitrate (LiNO3) are used as interface modifiers for high-energy LMBs. Based on the electrochemical analysis and density functional theory (DFT) calculations, it is verified that additives form well-designed LiF- and Li3N-based double-layer solid electrolyte interphase (SEI) on Li metal anodes.
Thereby, the designed electrolyte greatly improves the cycle performance of Li|LiNi0.8Co0.1Mn0.1 (NCM811) full cell at 606 cycles of 80.0% of capacity retention with stable coulombic efficiency (CE) higher than 99.5% at a charge reduction - switch-off voltage of 4.2V. Therefore, this new strategy paves the way to improve the energy density of LMBs with Ni-rich cathodes. Need for Li metal anodes and Ni-rich cathodes for high energy density batteries [1]. a) Li dendrite formation due to repeated volume expansion, (b) Continuous breakdown of reactive electrolyte and development of thick SEI layer [5]. Limits to the commercialization of Li metal anodes. a) Schematic formation of SEI and dead Li on the Li metal anodes during repeated cycles and hazards at Li metal anodes after contact with H2O and oxygen [6].
Schematic illustration of forming desirable CEI by LiDFBP [25]. a) Schematic representation of Li deposition after lithophilic additive deposition. TOF-SIMS 3D depth profile of Li metal anodes after 20 cycles in Li | NCM811 full cells with LiDFBP + LiNO3 electrolyte. Surface SEM images after plating on Cu substrate in 2016 coin, 0.5T spacer Li | Cu cells and expected mechanisms of Li plating with (a) LiDFBP and (b) LiNO3.
Electrochemical floating test of Li|NCM811 full cells in 6 hours at 4.2 V. After the pre-cycle, a 2nd charge was performed at a rate of 0.1 C to 4.2 V. a) Ni and Al dissolution measurement by ICP-OES.
Introduction of Li metal anodes and Ni-rich cathodes
Needs of Li metal anodes and Ni-rich cathodes
Problems of Li metal anodes
Problems of Ni-rich cathodes
Strategies for electrolytes of LMBs
Highly concentrated systems
Application of fluorinated solvents
Desirable CEI and SEI formation by electrolyte additives
In the case of highly reactive Li metal anodes, formation of thick organic SEI by salt or solvent improves electroconductivity, resulting in dendritic morphology, leading to short circuit, causing problems with the risk of cell explosion. Research is also being conducted to reduce the overvoltage in Li plating and to promote uniform Li deposition by adding the lithophile additive such as AgNO3, AgPF6 and AuNO3 to Li metal before Li deposition (Figure 11 ) [19]. Properties of inorganic SEI (LiF, Li3N and Li2CO3). a) Schematic representation of Li deposition after lithophilic additive deposition.
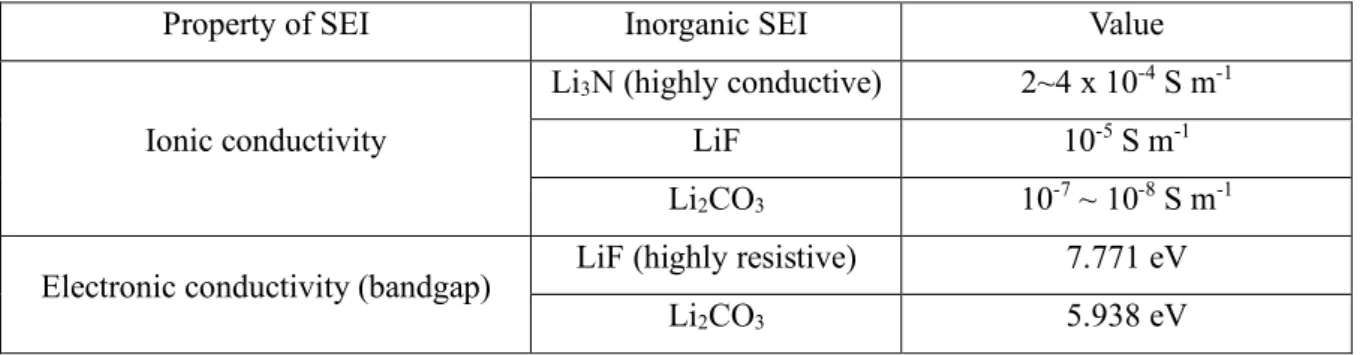
Experimental
Electrochemical measurements
2032 coin-type full cells with 100 μm foil-type Li metal anodes, PE separator and NCM811 cathode were fabricated in an Ar-filled glove box. After pre-cycling, the full Li|NCM811 cells were cycled at a constant current of 1.3 mA cm. For the electrochemical flow test, fully charged full cells (2nd charge at 0.1C rates up to 4.2V) have remained in constant voltage mode for 6 hours. 2) Li|Cu cells.
2016 coin-type cells with 100 μm foil-type Li metal anodes with 0.5T spacer, PE separator and Cu substrate were fabricated in an Ar-filled glove box. After pre-cycling, the Li|Cu cells were cycled through a battery measurement system (WonATech WBCS 3000) at a constant current of 1.3 mA cm-2 (corresponding to 0.5 C rates). 3) Li|Li symmetric cells. 2016 coin-type cells with 100 μm foil-type Li metal anodes with 0.5T spacer, PE separator and 100 μm foil-type Li metal anodes were fabricated in an Ar-filled glove box.
A pouch bag with 100 μm sheet-type Li metal anodes, PE spacers, and Al foil was fabricated in a padded glove box.
Characterization
Results and Discussion
DFT calculation and electrochemical performance of Li|NCM811 full cells
The oxidation and reduction trends of salts, DME and additives were calculated from DFT calculations (Figure 15). According to the LUMO energy level, the LUMO energies of the ionic additives showed that the reduction of LiNO3 occurs after the reduction of LiDFBP in Li metal anodes, noting that the difference of the LUMO energy level enables the formation of bilayer SEI, which is made of a single layer LiF-based inner and Li3N-based outer layer. Also, as shown by the HOMO energy levels, the +1e--DFBP- species generated by the reduction of the DFBP anion at the Li metal anode have the highest HOMO energy level, which develop LiDFBP-derived CEI at the NCM811 cathode , thereby increasing the oxidative stability of DME.
LiDFBP+LiNO3 electrolyte showed high capacity retention of 80% after 606 cycles at 0.5 °C temperature, while the capacity and CE without additive drop drastically around 45 cycles. electrolyte showed stable cycling performance and CE over 400 cycles, while the cell with LiDFBP electrolyte showed a sharp decline around 155 cycles. To check the reversibility of Li during repeated cycles, Li|Cu and Li|Li symmetric cells were tested. To realize a similar situation with full Li|NCM811 cells, the areal capacity was set to 2.6 mAh cm-2, which is the same for NCM811 cathodes.
Also, LiDFBP showed an increase in ICE compared to no additive, but it showed a lower ICE than LiNO3. To increase the initial plating voltage profile of LiDFBP and LiNO3, LiDFBP showed a slightly higher overpotential than LiNO3. Figure 19 also showed the cycling performance of Li|Li symmetric cells. showed a relatively low overpotential around 2500 h, while no additive showed a dramatic increase in overpotential around 450 h.
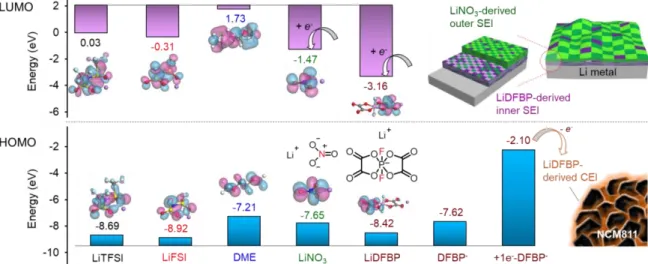
Effect of dual-layer SEI on the Li metal anodes
For each cycle, charge C rates were fixed at 0.5 C and discharge C rates were steadily increased from 0.5 C to 5 C every 3 cycles.
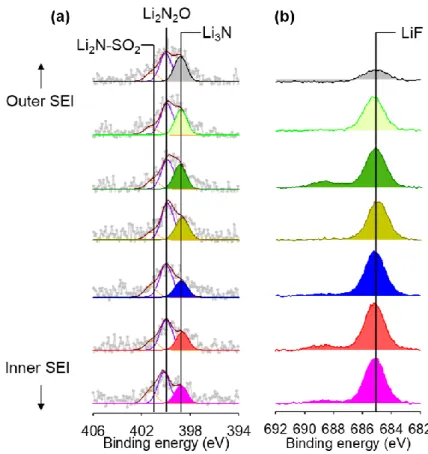
Effect of LiDFBP on the NCM811 cathodes
Each cell was pre-recycled with each electrolyte and stored in no additive ((2M LiFSI+1M LiTFSI) DME+1% LiDFBP+3% LiNO3) electrolyte for 1 day at 60℃. b) Schematic illustration of ICP-OES analysis. TOF-SIMS 3D characterization of NCM811 cathodes after 20 cycles with no additive and LiDFBP+LiNO3 electrolytes in Li|NCM811 full cells. TEM, FFT and EELS analysis of NCM811 cathodes after 60 cycles with no additive and LiDFBP+LiNO3 electrolytes in Li|NCM811 full cells.
Cross-sectional SEM images of NCM811 cathodes after 60 cycles without additive and LiDFBP+LiNO3 electrolytes in Li|NCM811 full cells.
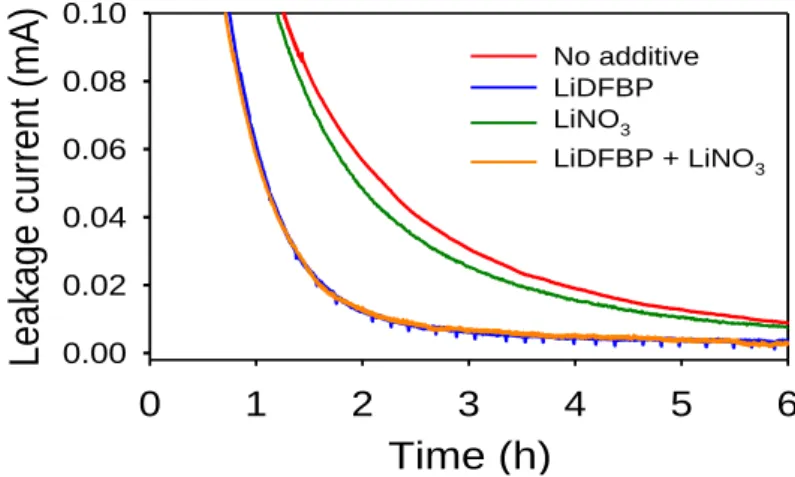
Conclusion
Osaka, Enhanced Cycling Performance of a Li Metal Anode in a dimethylsulfoxide-based electrolyte using highly concentrated lithium salt for a lithium-oxygen battery, Journal of Power Sources. Watanabe, Sulfolan-based high-concentration electrolytes of lithium-bis(trifluoromethanesulfonyl)amide: Ionic transport, Li-Ion coordination, and Li-S battery performance, J. Amine, cyclic carbonate for highly stable cycling of high-voltage lithium metal batteries, Energy Storage Materials.
Bao, Molecular design for electrolyte solvents enabling energy-dense and long-cycle lithium metal batteries, Nat. Choi, In situ interface tuning to achieve high-performance nickel-rich cathodes in lithium metal batteries, ACS Appl. Xu, Guided lithium metal deposition and enhanced lithium coulombic efficiency through synergistic effects of LiAsF6 and cyclic carbonate additives, ACS Energy Lett.
Qian, Dendrite-free lithium deposition by coating a lithophilic heterogeneous metal layer on lithium metal anode, Energy Storage Materials. Xiao, Research Advances in Understanding the Unique Interfaces between Concentrated Electrolytes and Electrodes for Energy.